RESEARCH
D. Y. Barsakcioglu, S. Luan, L. Grand, T. G. Constandinou
The era of bioelectronic healthcare is dawning upon us. As electronic systems shrink in size and improve in functionality, we see more and more emerging devices that can track vital signs, such as heart rate and blood pressure, realising the grand vision of highly connected sensor nodes monitoring patients’ health beyond the hospital doors. The real revolution in digital healthcare, however, lies in bringing not only the diagnostics but also the therapy to the patient which requires interfacing the world of electronics with biology.
Interfacing the nervous system provides an immense opportunity to observe (through recording) and modify (through stimulation) the functional state of the biological system to fundamentally understand various diseases and health conditions, and to ultimately develop suitable therapies through closed-loop systems [1]. Consequently, a host of neural interface modalities, with varying levels of invasiveness, have been developed over the past decades. Among all, interfacing at the individual neuron level allows the highest level of information transfer from the brain.
Despite the success of devices such as Cochlear Implants, interfacing at the individual neuron level is still severely limited due to challenges such as selectivity (for stimulation) and thermal-limitations imposed on data transmission to prevent neural tissue damage. The latter is a major bottleneck in improving information transfer rate of neural recording systems as they scale up. Hence, there is currently a tremendous drive to develop new enabling technologies for neuroscience to provide insightful views on how motor or sensory information is represented and transformed by the brain, as well as revealing how this complex system is affected by neurological injuries and disease.
The core mission of our research group, Next Generation Neural Interfaces – NGNI, is developing novel enabling neural interface technologies that target recording and stimulating thousands of individual neurons for fundamental neuroscience research and therapeutic medical devices.
Accessing the Brain
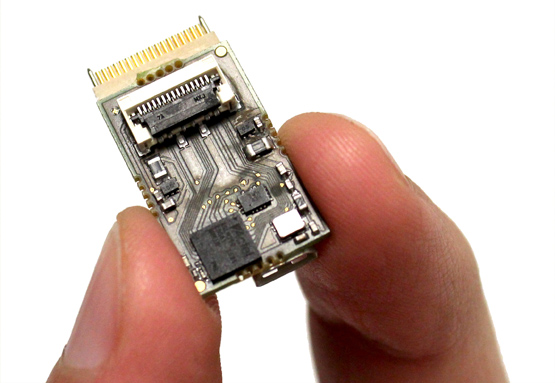
Figure 1. A 32 Channel NGNI headstage
Recent advances in micro- and nanofabrication methods are now enabling the design of neural interfaces with hundreds and even thousands of microelectrodes on the same implant. As a result, neuroscientists can realistically aim to capture the activities of thousands of individual neurons simultaneously. This dramatic leap in interfacing with neural circuits has introduced new questions and requires novel solutions for recording, analysing and transmitting the activities of large numbers of neurons, especially in real-time.
One of our key research objectives focuses on developing on-node spike sorting – associating spike events to individual neurons by classifying its features – solutions to reduce data dimensionality prior to transmission and achieve recording capabilities up to thousands of channels. At present, spike sorting is carried out with computers because of its high computational demands. This greatly limits portability and future expandability of the system. To fully leverage the benefit of high-channel count electrodes, we have developed a scalable, end-to- end solution for an on-node, real-time spike sorting platform. The lab ready version includes (1) a coin-sized 32 channel headstage under 2g of weight (Figure 1.A); (2) an electrically isolated USB 3.0 data acquisition card for safer in-vivo real-time data streaming, supporting maximum 32 headstages; and (3) a software suite for system control, data processing and visualization. The system also supports on-head- stage spike sorting, which reduces required bandwidth making wireless data transfer possible. This has been successfully verified in-vivo on a NHP model for real-time spike sorting. The next version will support automatic logging, wireless transfer and monitor up to several thousand channels. As the number of recording channels increases, more research and engineering effort should be invested to find solutions for high throughput data connection, neural signal compression, low power electronics and novel microelectrode design.
Targeting fully implantable high-channel count neural interfaces, our research group have also been developing various hardware efficient neural signal processing algorithms such as First and Second Derivative Extrema (FSDE) – a new feature – and Hierarchical Adaptive Means (HAM) – adaptive clustering, and novel integrated recording systems such as an ultra-low power neural signal processor. The developed 64-channel neural processor achieves an efficiency of 1.5 Giga operations per millijoule (mJ) which is several orders of magnitude better than off-the- shelf microcontrollers.
Closing the Loop
The capability of upper-limb prosthetics has leapt forward over the last decade, but even advanced devices still provide little to no feedback to the user, making them difficult to control without visual monitoring. To enhance prosthetic control and provide a naturalistic user experience, several initiatives such as HAPTIX aim to incorporate sensory feedback into upper-limb prosthetics [2]. Senseback is one such initiative among several universities in the UK.
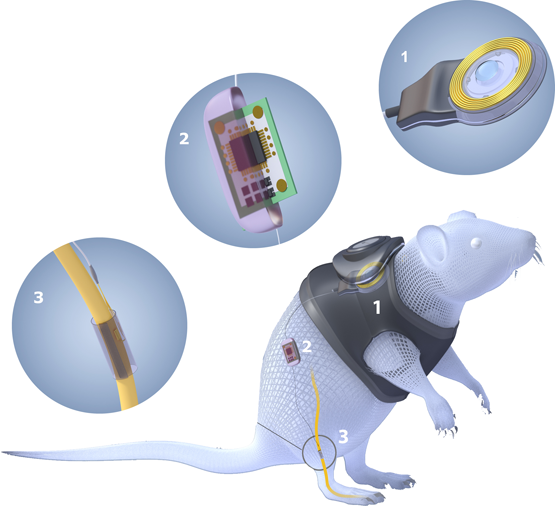
Figure 2. Project concept showing the three implanted devices. 1: power-data communication device. 2: Implantable stimulation and recording ASIC. 3: Silicon electrode array.
The goal of Senseback is to develop technologies that will enable the next generation of assistive devices to provide truly natural control through enhanced sensory feedback. To enable such feedback, we must meet two clear objectives: to generate artificial signals that mimic those of the natural arm and hand, and to provide a means of delivering those signals to the nervous system of a prosthesis user. To deliver technology addressing these objectives, Senseback team is developing implantable silicon probes & electronics, novel tactile sensors and biomechanical models; with key aspects of the technology validated in chronic in-vivo testing in a rat model.
At Imperial, we are developing chronically implantable electronics including a low frequency transcutaneous inductive link and a novel low power, low data rate bidirectional neural interface integrated circuit (IC). The highly flexible system will serve as a platform both for the Senseback project and beyond. One of the main challenges of the system being developed is to achieve selective stimulation capability. Currently, nerve-blocking experiments are being conducted in-vivo in a rodent model using sciatic nerve as an implant location with custom-made cuff electrodes.
Optogenetics: Illuminating the Future of Prosthetics?
Besides the typical and well established electrical stimulation principles, there exist a multitude of novel modalities for neuromodulation [3]. With the advancements in optogenetics [4], in which targeted gene regulators are introduced to make cells light sensitive, optical stimulation could be an effective alternative to electrical neuromodulation providing higher selectivity in terms of targeting specific neuron types and spatial selectivity, for example along the cortical column.
One such possible application of this technology is to focal epilepsy. NGNI is a key partner in a multi-site, cross-disciplinary project called CANDO – Controlling Abnormal Network Dynamics using Optogenetics – which aims to develop a cortical implant for optogenetic neural control and achieve the first-in- human trial in patients with focal epilepsy. The 7-year long project has now entered its third year and a fully functioning prototype of the neural implant has been implemented. The developed prototype is based on an array of active probes that can record local electrical activity and provide optical stimulus for neuromodulation. The various components of the system including the custom silicon probe design, recording instrumentation, stimulation optoelectronics, and closed-loop algorithm have been and are being validated in pre-clinical testing. This in-vivo data is essential in demonstrating both efficacy and safety, forming a crucial part of the technical documentation required for regulatory approvals in preparation for first-in- human trials planned for 2021.
Turning the Radar Towards the Brain
Another novel technology currently being developed by our group focuses on non-invasive functional neuroimaging. In collaboration with University of Oslo, we will use a single chip implementation of an impulse-radio ultra-wideband (IR-UWB) radar system to measure changes in regional cerebral blood volume. This project will deliver a wearable brain imaging device consisting of an array of antennas, and detect and locate brain activity non-invasively using digital beamforming techniques. The envisioned brain imaging device will be portable, low-cost, and will have sufficient temporal resolution to accurately track brain activity. This would allow for ambulatory assessment, as well as affordable neuroprosthetics.
The IR-UWB radar has been tested on human subjects to measure the heart rate proving that (1) one can get transmission through the head, and (2) one can measure the variations in blood volume within the brain. Having verified the initial hypothesis that one can measure the variations in intracranial blood volume using IR-UWB radar, the next step in the project is to test whether one can measure a functional signal using multiple radar modules in a 3D imaging array.
Future Directions
The era of bioelectronic healthcare is dawning upon us. As the boundary between human and silicon blurs, various therapeutic solutions for diseases and disabilities emerge. At the front-line of driving these technological break-throughs is the advances in neural interfaces. To sustain these advances and support the solutions of future decades, research into neural interfaces should strive for more compactness, power-efficiency, biocompatibility, and selectivity. This requires ever more increasing collaboration between neuroscience, material science, and electronic engineering.
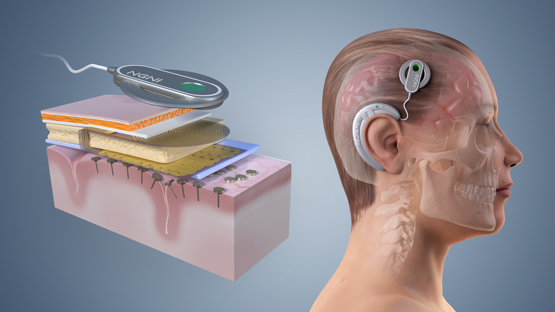
Figure 3. ENGINI Concept
Neuromodulation remains and will continue to be a key technology for therapeutic medical devices. On the other hand, motor control of prosthetics and assistive devices is still not clinically viable today due to a multitude of challenges including scalability of neural recording systems and stability of electrode-neural tissue interface. To address these grand challenges, NGNI group has embarked on a new ambitious project, Empowering Next Generation Implantable Neural Interfaces (ENGINI) that aims to deliver a novel neural recording interface consisting of distributed “neural recording pins” containing multiple recording channels and on-node neural signal processing.
As neural interfaces scale-up, we envision such distributed local processing to be pivotal. It may also be more efficient to relay higher level abstract information extracted from underlying neural signals which demand more processing at the sensor node. This is certainly a requirement for closed-loop applications which aim to deliver appropriate therapies via neural stimulation. Extracting such high-level information is a non-trivial task which may require incorporating concepts such as deep-learning and neuromorphic computing into the next generation of neural interfaces.
References
- Kristoffer Famm et al., “Drug discovery: a jump-start for electroceuticals,” in Nature, vol. 496, no. 7444, pp. 159 – 161, 2013.
- Emily L. Graczyk et al., “The neural basis of perceived intensity in natural and artificial touch,” in Science Translational Medicine, vol. 8, no. 362, 2016.
- Song Luan et al., “Neuromodulation: present and emerging methods,” in Frontiers in Neuroengineering, vol. 7, 2014.
- György Buzsáki et al., “Tools for probing local circuits: high-density silicon probes combined with optogenetics,” in Neuron, vol. 86, no. 1, pp. 92-105, 2015.
Authors
Dr. Deren Y. Barsakcioglu (S’10 – M’16) is a research associate in the Next Generation Neural Interfaces research group at Imperial. Following a B.Sc. degree in Electrical and Computer Engineering from University of Texas at Austin in 2010, Dr Barsakcioglu received M.Sc. and PhD degrees from Imperial College London in 2011 and 2016, respectively. Figure 3. ENGINI Concept
He has developed several feature extraction and spike sorting algorithms for hardware- efficient on-node neural signal processing. His current research involves developing autonomous and adaptive algorithms for on-node spike detection and sorting for long-term chronic monitoring of neural signals recorded from high-channel count neural interfaces. His main research interests include long-term chronic neural recording and biomedical signal processing, low-power embedded systems and machine learning.
Dr. Song Luan (S’08 – M’15) received the M.Sc. in analogue and digital integrated circuit design and Ph.D. degrees in biomedical microelectronics engineering from Imperial College London in 2010 and 2014 respectively. He works as a research associate in the Next Generation Neural Interfaces Lab.
He has designed different types of integrated neural stimulation circuits and systems with 0.35 and 0.18 um process. He is also an advanced engineer in PCB, firmware and software development for in-house custom hardware. His main research interests include chronic implantable neural interfaces and its applications, low power microelectronics and wireless power/data link.
Dr. Laszlo Grand received his MSc degree in Electrical and Computer Engineering and PhD in Neuroscience from the Pazmany Peter Catholic University in 2006 and 2010 respectively. He is a Research Officer in the Next Generation Neural Interfaces Lab and an adjunct Assistant Professor of the Johns Hopkins University School of Medicine Neurology and Neurosurgery.
He has developed various wire arrays and neural silicon probes for neurophysiological recording and stimulation, and methods for co-registering neurophysiological and behavioural data continuously 24/7. With his work on neuroscience he has contributed to the advancement of the epilepsy and traumatic brain injury fields. His main research interests include high-content imaging of neural cultures; implantable chronic closed-loop systems and biomedical data processing.
Dr. Timothy G. Constandinou (AM’98-M’01–SM’10) is a Reader in Neural Microsystems at Imperial College London and also Deputy Director of the Centre for Bio-inspired Technology. Dr Constandinou received BEng and PhD degrees in Electronic Engineering from Imperial College London in 2001 and 2005, respectively.
He leads the Next Generation Neural Interfaces research group at Imperial. The group utilizes integrated circuit and microsystem technologies to create advanced neural interfaces that enable new scientific and prosthetic applications. Within the IEEE and IET he serves on several committees/panels, etc, regularly contributing to conference organization, technical activities and governance. He is currently associate editor of IEEE Trans. Biomedical Circuits & Systems (TBioCAS), is chair-elect of the IEEE Sensory Systems Technical Committee, a member of the IEEE BRAIN Initiative Steering Committee, member of IEEE BioCAS Technical Committee, and recently been elected to the IEEE Circuits & Systems Society Board of Governors for the term 2017-19. He is currently chair of the IET Awards & Prizes committee and also serves on the IET Knowledge Services Board.